Protein Folding and Cellular Computation
- Dr. J. James Frost
- Apr 16, 2024
- 9 min read
Updated: Apr 20, 2024
In this second issue of BioMolecuar Insights Dr. Frost begins a tour of unrecognized and underrecognized areas for research and development in molecular imaging. The blinding pace of advancements in biology and molecular science can, at times, obscure new research opportunities as lab directors, investigators, and students remain deeply imbedded within their own projects.
As Dr. Frost states, “When I was running a busy lab, applying for funding, writing papers, and mentoring students, it was difficult to look up and glance outside at what was going on around me”.
In this issue of BioMolecular Insights, Dr. Frost first considers the foundational biologic process of protein folding and new opportunities in biomolecular imaging, from dementia to cancer. Next, Dr. Frost explores a topic in biologic computational intelligence where he highlights a recent publication in the physics literature that examines the limits of any biological organism to predict and plan for uncertain futures. That publication focuses on the foundational physical parameters of energy, time and noise filtering.
This builds on the Trilogy Dr. J. James Frost has written on cancer’s computation featured here https://www.drjjamesfrost.com/publications.
The newsletter article goes on to explore the limits and conditions for biological computation across the spectrum from higher to lower life forms – perhaps down to the individual cell. Dr. Frost challenges the reader to examine this interesting topic in light of human decision making and our own planning for uncertain futures. Completing the circle, the relevance to cancer and its treatment is addressed.
Protein Folding
The Magnitude of the Problem
The ability of long protein strands to fold into biologically active structures has long fascinated biologists, physicists, and mathematicians. Early on, it was thought that proteins folded in to functional 3-D structures by amino acids binding to each other sequentially by trial and error until the correct structure was formed. Yet, the number of steps and the necessary time is prohibitive. For a short 100-mer protein the number of steps is about (100-1)! = (99·98·97···), equal to 10^156. Assume each amino acid pair interaction takes 1 picosecond, then the time to fully form a 3-D structure is would be 10^144 seconds. The age of the universe is approximately 10^17 seconds and therefore there is not enough time in the universe to permit the protein to fold with the trial-and-error mechanism. The problem is exponentially greater with longer protein strands of biological relevance. In 2021 Science Magazine awarded the Breakthrough of the Year to artificial intelligence computational algorithms for the prediction of protein structures.
It is now recognized that a family of auxiliary proteins called chaperones directly aid and promote correct protein folding. Without the chaperones, the protein sequences would never form into functional 3-D structures. The system of chaperone proteins and their actions is known as the Unfolded Protein Response or the UPR. Some of the proteins are XBP1, ATF6, eIF2α, PERK, IRE1 and GRP78. The heat shock proteins, such as HSP90, are also involved in protein folding.

The bulk of protein folding occurs at the endoplasmic reticulum, ER; “ER stress” occurs when the UPR is overloaded or otherwise compromised. The UPR chaperones act in a defined sequence as the protein folding process progress to the final 3-D structure. The UPR constituents control protein entry into the ER, ER biogenesis, ER degradation (ERAD) and other processes.
The UPR also plays a role in processes other than folding, including lipid and energy metabolism, innate immunity, and autophagy. Information about protein folding is transmitted back to the nucleus to control unfolded protein flux through the nuclear pores (a topic for a future BioMolecular Insights piece). Adding further complexity, mitochondria have their own folding system: the UPR-MT. In short, the UPR is critical for cell survival and growth.
Maladaptive Protein Folding in Dementia
The protein folding abnormalities of Alzheimer disease (AD) are well known. Abnormally folded beta-amyloid and tau proteins accumulate in the brain and are thought to lead to AD. This theory is the stimulus for the enormous effort by the pharmaceutical industry to develop drugs that counteract and reverse abnormal protein accumulation in the brain. In the earliest pre-clinical stages of the disease the UPR and ER stress play a role in the slow evolution to frank misfolded protein brain deposition.
Early in the process ER stress is reversible through UPR adaptation, but later becomes irreversible. The inability of the UPR to control accurate protein folding and subsequent ER stress leads to a cascade of subsequent events that result in clinical AD. In Parkinson disease a similar process occurs; the alpha-synuclein Lewy bodies are the causative misfolded protein.
Yet, we know comparatively little about the early folding events and how to detect and control them. Early research has indicated that the UPR and ER stress can be modified with pharmacologic agents, possibly ameliorating or normalizing nascent misfolding disturbances. One focus is the upregulation and activation of HSPA5 and PERK-eIF2α signaling to ameliorate ER stress and restore homeostasis. Another highlights the role of PTEN and the ER stress markers HSPA5 and DDIT3. Yet, there is presently no non-invasive technology to examine the dysregulated components of the brain UPR in degenerative disease. The initial folding abnormalities must be detected at an early stage, including the specific molecular components of the UPR that are dysregulated. This is where biomolecular imaging could play a pivotal role.
Enhanced Protein Folding in Cancer
In cancer, the situation is reversed. Rapid cell growth in cancer requires an immense increase in the synthesis of many cellular constituents, including, prominently, proteins. This, in turn, leads to a large increase in UPR activity and a requirement to control ER stress. Adaptive mechanisms in cancer permit ongoing control of cancer cell growth. Without the ability of proteins to correctly fold into functional 3-D structures, growth would be arrested.

The UPR is increasingly viewed as a druggable target for cancer therapy. Upregulation of the UPR components referenced above is now recognized, offering a chemotherapeutic strategy directed at inhibition. Correspondingly, new drugs and many natural substances, are being developed to inhibit GRP78 expression, eIF2α phosphorylation, XBP1 splicing and several other UPR components.
These agents have been successful in otherwise nonresponsive tumors, including gastric, breast, myeloma, colorectal and pancreatic cancers, among several others. Importantly, targeting the UPR in cancer targets a mechanism across all cancers and thus would have widespread applicability. Again, no noninvasive technology currently exists to identify, target, and monitor the UPR in cancer. Yet, where the therapeutic drugs exist, the specialized imaging probes need not be far behind.
The Path to UPR Imaging
Even with this brief introduction to protein folding, one will quickly perceive the many potential applications for molecular imaging. Importantly, a review of the scientific and patent literature indicates the existence of high affinity molecules needed as noninvasive imaging probes. In cancer, one could detect the precise upregulated components of the UPR and then specifically target for inhibition. The degree of inhibition of the UPR could then be subsequently monitored with imaging, redirecting treatment if the inhibition and consequent therapeutic response is inadequate. There are many others, of which cancer biologists, oncologists, and imaging clinicians are well aware – with protein folding-based diagnosis and therapy one is simply changing the targets to those at bottom in the cancer growth and dissemination process.
In the case of dementia, caused or exacerbated by the deposition of misfolded proteins in the brain, the uses of UPR biomolecular imaging are, as with cancer, many. Yet, whereas in cancer one desires to inhibit protein folding, in dementia one aims to enhance correct folding. This goal is paramount at the very earliest stages of misfolding and ER stress before an irreversible cascade of protein misfolding ensues. At present we have no noninvasive imaging tools to detect and monitor the UPR and ER stress in the brain. Yet, the strategies referenced above for cancer are eminently applicable to the brain UPR. As with other targets, the desirable probe properties for imaging in the brain and periphery are different, yet the basic strategy is the same. Noninvasive detection of abnormalities in the UPR in at-risk individuals or those with MCI could well lead to new and beneficial therapies, as before, that could be then also monitored with imaging.
The UPR includes a rich array of targets for molecular imaging, at present unaddressed and untapped. In cancer, new therapeutic advances already target the UPR. This approach will continue to advance as new drugs are developed for the myriad of UPR chaperones. In the brain, UPR drug targets are less far along, but the case is just as compelling and perhaps more so coupled with amyloid and tau protein-reducing drugs currently available. As always, the challenge for the imaging community will be the identification and development of the high affinity molecules required for in vivo imaging. An initial review of the scientific and patent literature demonstrates that they do exist. In the cases where they don’t, our medicinal and radiochemists will meet this challenge, as they have for the past 40 years since the advent of non-invasive molecular imaging.
Intelligence and Future Prediction in Lower Lifeforms
In my article Cancer’s Intelligence I conjectured that cancer is able to compute survival solutions to future environmental changes that it has not yet encountered. That is, that cancer can predict the future and ready itself to adapt to new, yet unseen environmental conditions, whether a change in oxygen tension or a chemotherapeutic drug.
A recent, exceedingly relevant paper entitled "Trade-offs between cost and information in cellular prediction" by Tamla et al analyzes cellular adaptation and lays out the conditions for prediction of a survival response to an adverse environmental change. The cellular response is an intrinsically computed solution to future external events using the cell’s internal computational machinery. Historically, it has been thought that only higher organisms “can predict the future environment and initiate a response ahead of time”, but this is not the case.
Energy and Time
At bottom, energy and time are two key computational limits. Energy (free energy) for computation, storing information, and the protein synthesis needed for future actions limits computation of the optimal solution for future survival moves. Time is a factor in computing solutions and a in sensing and sampling the current and past environments sufficiently finely for accurate predictions. Optimal time averaging for the cell is key, which also has the advantage of filtering noise.
The Tjalma article elucidates the informational bounds a cell can possibly have about the future. The costs of a bit of informational sampling and processing are computed and compared. As information-processing humans, we extract only the most useful information from the past environment as we plan future actions – the cell does the same. How and under what conditions a cell approaches the information bound for its internal computation is examined in detail by exploring Markovian and non-Markovian models and by examining the necessary fineness of temporal sampling of the environment and the cell’s computational speed. The information bottleneck theory is key in this analysis, as it dictates information compression for storage and its costs.
Cancer’s Predictive Engine
The article is a challenging, yet rewarding read and one that is intimately related to the vast computation ability of the cancer cell and more importantly, cancer cell networks. In a way, this article is the missing link between cancer’s computation from instantaneous, present environmental conditions – the constrained regime held by most cancer biologists – to the tracking and selective sampling of the past and the present to the response to yet-unseen future events, just as human and higher animal computation occurs.
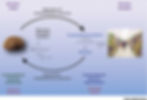
One can imagine several next steps extending from the consideration of the computational bounds for cell networks in which cells sense and transfer information among the network nodes and simultaneously sense and respond to the environment. In cancer, the combinatorial space of the vast tumor genotypic and phenotypic heterogeneity increases cancer’s computational state space enormously – accounting for cancer’s ability to escape the majority of lethal moves against it. Cancer’s choice to move or grow (epithelial-mesenchymal transition) and the advance construction of the metastatic niche well prior to the movement of the first metastatic cell are paramount unsolved problems of cancer’s lethal march. How many solutions to uncertain future environments can a cancer cell and its intercellular network ultimately store? Humans, of course, store multiple future scenarios and actions with probabilities and bet hedging within a game theoretical framework.
Cancer’s Computational Network
Next, if the molecular mediators of cancer’s tremendous computational potential could be elucidated, then they could be targeted and possibly degraded. This could, in turn, render cancer less able to out-compute the lethal moves by the oncologist. What is actually the computational backbone of cancer: the whole cell and interaction network or a substructure more amenable for targeting? The enhanced broken symmetry of cancer in its computational network is another relevant factor. See the first article in my cancer physics trilogy: Symmetry and symmetry breaking in cancer: a foundational approach to the cancer problem. Network sites of reduced symmetry are weak and thus more amenable to network disruption or destruction.
This exciting and extraordinarily detailed paper by Tjalma et al could provide the theory and practical algorithms for a new stage of understanding and manipulating intrinsic cellular computation, with far reaching implications for solving the cancer problem and providing benefit to our patients. This is the work that I have been seeking for some time.
For more information on the molecular imaging aspects of cancer characterization, treatment, and monitoring, please visit BioMolecular Imaging, LLC http://biomolecularimaging.com
Direct Contact
E-mail: DRJJAMESFROST@GMAIL.COM
Website: www.DrJJamesFrost.com
Twitter: www.twitter.com/drjjamesfrost
Facebook: www.facebook.com/drjjamesfrost
SoundCloud: www.soundcloud.com/drjjamesfrost
ORCHID ID: www.orchid.org/0000-0002-5148-6560